The potential for hydrogen embrittlement starts with the ingress of hydrogen into the metal. When hydrogen is in molecular form (H2), it cannot enter directly into the metal since the hydrogen molecule size is greater than the ‘free volume’ between metal atoms. Consequently, hydrogen can only enter into metals that can catalyze the molecular hydrogen to dissociate into individual hydrogen atoms (H) on the metal surface. These hydrogen atoms can then enter and penetrate (diffuse) further into the metal by occupying the free volume between metal atoms. During this diffusion process hydrogen atoms can encounter metallurgical defects, and it is these hydrogen-defect interactions that lead to material degradation. These degradation events at the micro-scale are recognized as hydrogen embrittlement at the macro-scale by the formation of cracks. Such cracks can then propagate through the pressure boundaries of hydrogen containment components, leading to unintended hydrogen release. Since the dissociation of molecular hydrogen and diffusion of atomic hydrogen are time-dependent in nature, the formation and propagation of cracks are similarly time-dependent.
While the terms ‘hydrogen embrittlement’ and ‘material compatibility’ are often invoked interchangeably, the phenomenon of hydrogen embrittlement is not solely a material compatibility issue. This perspective is illustrated from the diagram in Figure 1, in which the circles represent three types of variables: material properties, environmental conditions, and mechanical stress characteristics. Hydrogen embrittlement is activated at the intersection of these three variable types, indicating that the phenomenon depends not only on material properties but also on environmental conditions and mechanical stress characteristics. In practical terms, a metal comprising the pressure boundary in a hydrogen containment component may function safely under one combination of environmental conditions and mechanical stress characteristics, but this same metal may suffer hydrogen embrittlement under a different combination of these variable-types.
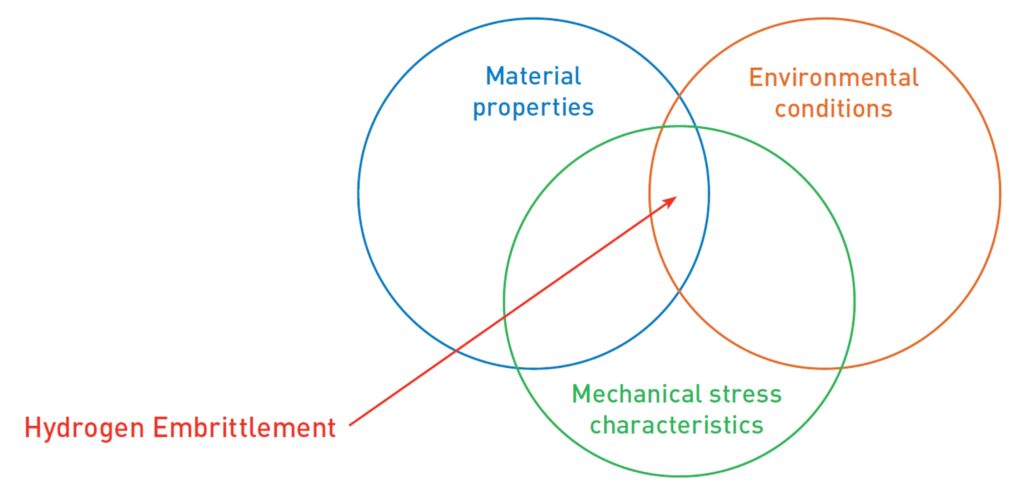
Although the diagram in Figure 1 is qualitative, it can still serve as a guide to identify variables that must be considered when assessing the risk for hydrogen embrittlement. In the material properties space, variables such as material strength, alloy-element composition, and metallurgical features can all affect the potential for hydrogen embrittlement. One prevailing trend across all classes of metals is that higher-strength alloys are more prone to hydrogen embrittlement than lower-strength alloys. In the context of Figure 1, this means that hydrogen embrittlement can be activated in higher-strength alloys for more combinations of environmental conditions and mechanical stress characteristics compared to lower-strength alloys. A practical extension of this concept is that managing hydrogen embrittlement by modifying environmental conditions or mechanical stress characteristics may be less effective for hydrogen containment components fabricated from higher-strength alloys. One example of a higher-strength alloy that can be encountered in components employed for hydrogen containment is 17-4 PH stainless steel. There are documented cases of components fabricated from 17-4 PH stainless steel that have failed in gaseous hydrogen service.1
In the mechanical stress characteristics space in Figure 1, one important consideration is the time-variation of mechanical stresses on metals in hydrogen containment components. Specifically, the activation of hydrogen embrittlement can depend on whether the applied mechanical stress is constant or whether it repeatedly cycles between maximum and minimum levels. For example, as mentioned previously, lower-strength alloys tend to be more resistant to hydrogen embrittlement than higher-strength alloys. However, this trend is most consistent when applied mechanical stresses are constant. Certain lower-strength alloys may not exhibit hydrogen embrittlement under constant mechanical stress, but when the same alloys are subjected to cyclic mechanical stress, then hydrogen embrittlement may be promoted. In addition to the time-variation of mechanical stresses, the absolute magnitudes of mechanical stresses can dictate the activation of hydrogen embrittlement. In particular, geometric discontinuities in containment components can give rise to elevated mechanical stresses, and in turn these sites promote the formation of hydrogen embrittlement-related cracks. A real-world case study of how mechanical stresses can govern the activation of hydrogen embrittlement is the spate of failures that occurred in steel hydrogen transport cylinders throughout Western Europe in the 1970s. Two prominent factors that contributed to these hydrogen embrittlement-related failures were cyclic mechanical stresses resulting from filling/discharging operations and localized mechanical stress concentration at the base of the cylinders.2
The final space in Figure 1 is environmental conditions, and here two of the primary variables influencing the activation of hydrogen embrittlement are gas pressure and temperature. As hydrogen gas pressure increases, hydrogen ingress into metals becomes more extensive so the potential for hydrogen embrittlement is enhanced concomitantly. Such enhanced hydrogen embrittlement susceptibility does not continue indefinitely as gas pressure increases since the severity of hydrogen-induced material degradation reaches a limit. Regarding temperature, the potential for hydrogen embrittlement is typically highest at near-ambient conditions. As temperature decreases, hydrogen diffusion becomes slower, which can limit the extent of hydrogen penetration into metals. At higher temperature, although hydrogen diffusion is accelerated, there is less interaction between hydrogen and metallurgical defects. As a result, at both lower and higher temperatures, hydrogen-induced material degradation is less severe.
The high-level view encapsulated in Figure 1 can guide various approaches for assessing the risk of hydrogen embrittlement in hydrogen containment systems. In some scenarios, simply identifying key variables associated with a hydrogen containment component may be sufficient to assess the risk of hydrogen embrittlement. For example, a component fabricated from lower-strength steel and subjected to constant mechanical stresses at typical design levels is unlikely to suffer hydrogen embrittlement.
Another way the concepts in Figure 1 can be helpful is when the successful field experience of hydrogen containment systems serves as a reference for evaluating the hydrogen embrittlement potential in other systems considered for hydrogen service. Here, the guidance from Figure 1 is to determine whether the three variable types associated with the system in question may enhance the potential for hydrogen embrittlement relative to the system with successful field experience. For example, assume the two systems are identical in the material properties and environmental conditions spaces, but the system with successful field experience was only subjected to constant mechanical stresses while the system in question is expected to experience cyclic mechanical stresses. In this case, the successful field experience cannot provide confidence that the system in question is safe from hydrogen embrittlement.
Similar reasoning must be applied in circumstances when hydrogen embrittlement potential in containment systems is evaluated based on data from materials testing in hydrogen gas. In this approach, it is imperative that properties of materials as well as environmental conditions in the testing relate to the hydrogen containment system in a conservative fashion. One specific example of the need to apply materials testing data in this judicious manner is represented by the 300-series stainless steels. For these steels, the potential for hydrogen embrittlement is highest at temperatures just below typical ambient conditions. Thus, in a containment system fabricated from 300-series stainless steel that operates at -50°C, the risk of hydrogen embrittlement cannot be reliably evaluated based on testing this steel in hydrogen gas at room temperature.
In summary, when assessing the potential for hydrogen embrittlement in containment systems, it is essential to consider the three variable types featured in Figure 1. Although there are numerous specific variables that populate the spaces depicted in Figure 1, the following list represents one variable from each space that deserves particular attention:
- Material strength. When procuring containment components that are not explicitly certified for gaseous hydrogen service, it is advisable to review the list of materials in the product drawing to identify any higher-strength alloys, such as 17-4 PH stainless steel, that raise concerns for hydrogen embrittlement.
- Time-variation of mechanical stresses. When containment components are subjected to cyclic mechanical stresses, the most effective means to account for hydrogen embrittlement is through quantitative analysis. These methods couple stress analysis of components with data from materials testing in hydrogen gas. Examples of such approaches are in the American Society of Mechanical Engineers (ASME) codes for stationary pressure vessels (ASME Boiler and Pressure Vessel Code, Section VIII, Division 3, Article KD-10) and for pipelines (ASME B31.12).
- Hydrogen gas pressure. For many metals, hydrogen embrittlement can be activated in gaseous hydrogen even at very low pressures (for example, 1 bar or less). For this reason, it cannot be assumed that there is a threshold pressure below which containment systems are safe from hydrogen embrittlement for all combinations of material properties and mechanical stress characteristics.
About the author
Dr. Brian Somerday is currently a Materials Engineering Consultant with Somerday Consulting, LLC., a member of the Hydrogen Safety Panel, and routinely supports activities at the Center for Hydrogen Safety. He has 25 years of experience in mechanical metallurgy with a focus on environmental effects on fracture and fatigue of structural alloys. Brian has published extensively on the topic of hydrogen-assisted fracture and fatigue in structural alloys, including co-authoring the Technical Reference for Hydrogen Compatibility of Materials and co-editing the two-volume set Gaseous Hydrogen Embrittlement of Materials in Energy Technologies (Woodhead Publishing, 2012).
About the CHS
Founded in 2018, the Center for Hydrogen Safety (CHS) is a non-profit, unbiased, corporate membership organization promoting the safe operation, handling, and use of hydrogen and hydrogen systems across all installations and applications. CHS has more than 100 global member organizations and 14 strategic partners and utilizes best practices, lessons learned, education resources, conferences, webinars, workshops, and working groups to develop and share hydrogen safety knowledge.
A trusted and highly respected resource, the Hydrogen Safety Panel (HSP) is a pioneer in reducing knowledge barriers to hydrogen fuel cell deployment and enabling timely technology adoption by cities and communities. Building on its diverse knowledge, rich experience, and technical objectivity, this not-for-profit expert panel utilizes safety reviews, research, information dissemination, and training to help government agencies, industry and other stakeholders ensure that hydrogen is safely stored and handled.
Opening image: Hydrogen Embrittlement, also known as Hydrogen Induced Cracking (HIC). Image © Uwe Aranas, dreamstime.com